Exoplanets orbiting dead stars: a future for Earth?
The universe holds many mysteries, and one fascinating area of study is the existence of exoplanets that orbit dead stars, specifically white dwarfs.
As we consider Earth’s future, it’s essential to explore how these celestial bodies might thrive long after their stars have extinguished.
Current research indicates that the sun will transform into a red giant in approximately 6 to 7 billion years, ultimately shedding about 50% of its mass as it becomes a white dwarf.
Recent observations from cutting-edge instruments like the James Webb Space Telescope have shed light on this captivating phenomenon, revealing that two out of four studied white dwarfs harbor large exoplanets.
With estimated ages of 5.3 billion and 1.6 billion years, these white dwarfs have been in their current state for several hundred million years, posing vital questions about the survival of planets as their stars evolve.
Given that our solar system may eventually mirror these distant systems, these insights can provide a glimpse into the potential for Earth and similar planets to endure the aftermath of stellar evolution.
Understanding the Lifecycle of Stars
The lifecycle of stars is a fascinating journey that begins in nebulae, vast clouds of gas and dust. From these primordial materials, stars emerge as protostars, gradually increasing their temperature and pressure until nuclear fusion ignites, marking the start of their main sequence phase. Stars spend about 90% of their lives in this stable period, where they maintain a balance between gravitational collapse and nuclear fission. For instance, our Sun, having existed for approximately 5,000 million years, is halfway through its estimated 10 billion-year lifespan.
As stars evolve, they transition through various stages. Once they exhaust the hydrogen in their cores, they begin to swell into red giants, increasing significantly in size. Red giants can reach dimensions 20 to 100 times that of our Sun, while their mass may only be 0.25 to 8 times greater. During this time, they also heat up, exhibiting surface temperatures between 4,000 K and 5,000 K, and eventually lead to intricate phenomena such as planetary nebulae.
This transition highlights an essential aspect of stellar evolution and its potential impact on surrounding exoplanets. As stars expand and shift in brightness and temperature, they can significantly alter the conditions necessary for planetary habitability. Some exoplanets orbiting these aging stars may find themselves in hostile environments as radiation increases, particularly from M dwarf stars, which emit radiation that can be 80 to 500 times more intense than our Sun.
Following the red giant phase, stars conclude their lifecycles by either becoming white dwarfs, experiencing supernovae, or collapsing into neutron stars. Roughly 6% of the stars in the Milky Way are classified as white dwarfs, which shine dimly at only 0.1% to 1% of the Sun’s brightness. Their development is critical for understanding the fate of existing exoplanets that may persist beyond their stars’ active lives. The end of a star’s lifecycle serves as a gateway to new possibilities, often affecting the prospects for surrounding celestial bodies.
From Main Sequence to Red Giant
The journey of a star begins in the main sequence phase, where it spends about 90% of its total lifetime fusing hydrogen into helium. This stage varies significantly based on the star’s mass. For instance, O5 spectral type stars with around 40 solar masses have a main-sequence lifetime of about 1 million years, while M0 spectral type stars, which are much smaller, can last up to 200 billion years.
As stars evolve and exhaust their hydrogen fuel, they transition to the red giant phase. At this point, the core becomes significantly hotter, prompting an increase in helium generation. The envelope of the star expands dramatically due to hydrogen shell burning. This expansion can lead to the incineration of inner planets, while many outer planets may endure the transformation.
Red giants, typically ranging in mass from 0.3 to 8 solar masses, exhibit surface temperatures around 5,000 K or lower, making them cooler than their predecessors. These stellar giants can reach luminosities nearly 3,000 times that of the Sun, alongside a radius that can expand to about 200 times that of our star. While the red-giant phase for a solar mass star lasts roughly 1 billion years, stars with masses greater than 8 solar masses may instead produce supernovae after their main sequence phase.
Spectral Type | Mass (Solar Masses) | Main Sequence Lifetime (Years) | Characteristics during Red Giant Phase |
---|---|---|---|
O5 | 40 | 1 million | Short-lived, intense fusion |
B0 | 16 | 10 million | Luminosity increases significantly |
A0 | 3.3 | 500 million | Expands to red giant, outer planets may survive |
F0 | 1.7 | 2.7 billion | Visible brightness increases |
G0 (Sun) | 1.1 | 9 billion | Expected to become a red giant in 5 billion years |
K0 | 0.8 | 14 billion | Long-lived, temperature decreases |
M0 | 0.4 | 200 billion | Possible longevity beyond the universe’s current age |
The transformation from main sequence to red giant highlights critical stellar phases that shape the universe. Understanding these transitions offers insights into the fate of stars like our Sun and the celestial dynamics around them.
What Happens After a Star Dies?
The cycle of a star culminates in a dramatic transformation known as stellar death. As a star exhausts its nuclear fuel, it initiates a series of processes that can lead to the star shedding its outer layers, ultimately resulting in a remnant such as a white dwarf. The fate of surrounding planets during this profound change introduces various outcomes based on their proximity and characteristics.
During the later stages of a star’s life, particularly when it expands into a giant phase, the immense size brings about destructive forces that may obliterate nearby planets like Mercury and Venus. As the star tackles extreme changes, its size can increase by hundreds of times, creating conditions inhospitable for any close orbiting bodies.
After the star has completed shedding its outer layers, what remains is a dense core—a white dwarf. The presence of this remnant has significant implications for the survival of planets lingering in its gravitational influence. Studies have identified various factors that contribute to a planet’s ability to endure these tidal effects:
- Distance: A planet’s distance from the white dwarf significantly determines its fate. A white dwarf that is 3 billion years old poses a different survival challenge than one that is 5 billion years old.
- Viscosity: The internal viscosity of a planet plays a crucial role in its resilience against gravitational forces. More viscous planets are better equipped to endure the chaotic environment.
- Size: Smaller planets generally experience reduced gravitational pull towards the remnant, increasing their chances of survival compared to larger planets.
Research indicates that Earth-like planets could exist in stable orbits around a white dwarf for substantial durations, capable of surviving for hundreds of millions of years. However, current observations predominantly reveal the presence of giant planets and small asteroids orbiting these remnants. There remains a notable absence of terrestrial-like planets in these observations.
Despite stellar death leading to chaos and destruction, the remnants can also serve to create conditions favorable for the formation of new planetary systems in the distant future. This fascinating dynamic underscores the complex interplay between life and death in the cosmos.
Factor | Impact on Planet Survival |
---|---|
Distance from White Dwarf | Closer proximity increases destruction risk. |
Internal Viscosity | Higher viscosity allows better stress resistance. |
Planet Size | Smaller size leads to reduced gravitational pull. |
Age of White Dwarf | Younger white dwarfs are more favorable for surviving planets. |
Characteristics of White Dwarfs
White dwarfs are fascinating stellar remnants formed from stars that have exhausted their nuclear fuel. These compact objects possess unique characteristics that distinguish them in the cosmic landscape. Over 95% of stars will eventually become white dwarfs, showcasing their significance in the life cycle of stars.
With typical masses ranging from 0.17 to 1.33 times that of the Sun, white dwarfs usually exhibit a peak mass around 0.6 M☉. The maximum mass a non-rotating white dwarf can attain, known as the Chandrasekhar limit, is approximately 1.44 M☉. This limit underlines the intricate balance of gravitational forces and electron degeneracy pressure that governs these stellar remnants.
One remarkable feature of white dwarfs is their extraordinary density, often reaching between \(10^4\) and \(10^7\) g/cm³, making them about 1,000,000 times denser than the Sun. For reference, the estimated density of a typical white dwarf is roughly 1,000,000 kg/m³, leading to a radius that is only approximately 0.8–2% of the Sun’s radius.
The cooling process of white dwarfs is a slow and gradual affair. The oldest known white dwarfs still radiate at temperatures of a few thousand kelvins, highlighting their longevity. Calculations indicate that cooling into a cold black dwarf would take longer than the current age of the universe, around 13.8 billion years.
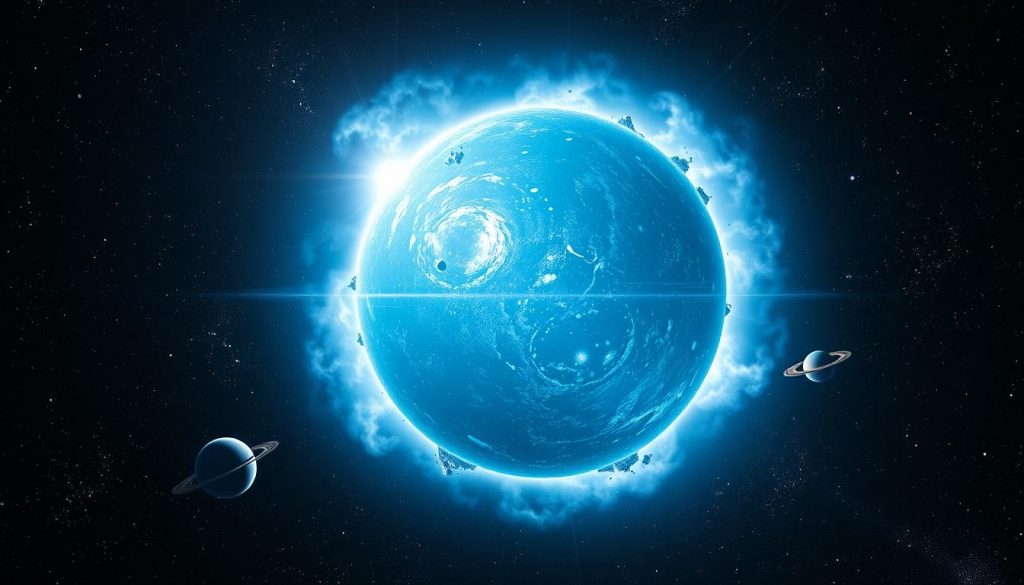
Observational characteristics further enhance the importance of white dwarfs. A significant portion of these remnants, nearly 4%, with dust disks, also show gaseous components. Currently, about a dozen white dwarfs with gaseous disks have been identified, while over 110 candidates signal the existence of disks through infrared excess. Spectroscopic studies have revealed the presence of over 50 emission lines in some, indicating the complex interactions occurring within these systems.
Characteristic | Value |
---|---|
Typical Mass Range | 0.17 M☉ to 1.33 M☉ |
Peak Mass | 0.6 M☉ |
Chandrasekhar Limit | 1.44 M☉ |
Density | 104 to 107 g/cm³ |
Surface Temperature | A few thousand kelvins |
Estimated Cooling Time | Longer than 13.8 billion years |
The study of these stellar remnants offers insights into the broader mechanisms of stellar evolution and the potential for exoplanets in their vicinity. The presence of heavy elements in their atmospheres hints at the complex interactions with surrounding planetary systems, further emphasizing the relevance of white dwarfs in contemporary astrophysics.
Exploring Exoplanets Orbiting Dead Stars
The fascinating world of exoplanets orbiting dead stars, particularly white dwarfs, has garnered significant interest among astronomers. White dwarfs serve as remnants of small- to medium-sized stars after they have exhausted their nuclear fuel, presenting unique conditions for celestial bodies nearby. Observational advances, notably through the James Webb Space Telescope, allow scientists to better detect and analyze these exoplanets, providing insights into their atmospheres and potential for life.
White dwarfs possess significantly smaller sizes and featureless characteristics. This configuration enhances visibility for transiting terrestrial planets, making it easier to examine their atmospheres in greater detail. The evolutionary stages of stars often involve dramatic physical changes. For instance, as these stars expand to around 100 times their normal radius during the first destruction phase, they may engulf nearby planets. This significant alteration in size can lead to extreme temperatures and conditions that potentially evaporate any oceans present on these planets.
To maintain appreciable water levels during this expansion, a planet must initially reside approximately 5 to 6 astronomical units from its dying star. In contrast, after the star transitions into a white dwarf and cools over a billion years, the required distance for liquid water to exist narrows down to about 1% of 1 astronomical unit. Such drastic changes highlight the challenges for exoplanets in maintaining stability and habitability.
Tidal migration can play a role in altering a planet’s orbit, shifting it closer to a white dwarf. While this process may lead to a stable orbit, it presents risks. The journey to remain in a habitable zone is described as a perilous one, emphasizing the difficulties some planets face in surviving the dynamic changes associated with their stars. Ongoing research is crucial to improve our understanding of white dwarf-planet systems, ultimately enhancing the probability of identifying exoplanets with hospitable conditions.
Case Studies of Notable Exoplanet Discoveries
The exploration of exoplanet discoveries has revealed fascinating insights into the nature of planetary systems near white dwarfs. These findings, backed by extensive observational data, highlight the resilience of certain planets during the tumultuous life cycles of their parent stars. Among significant case studies, 51 Pegasi b stands out as the first confirmed exoplanet around a main-sequence star, a discovery that paved the way for understanding the variety of planetary systems.
Another noteworthy example, PSR B1620-26 b, is recognized as the oldest known extrasolar planet, boasting an estimated age of 12.7 billion years. This case study offers a unique perspective on how planetary systems can endure beyond the typical lifespan of standard stars. Moving to the realm of gas giants, the discovery of Upsilon Andromedae ensured the classification of multiple adjacent exoplanets, featuring Jupiter-like masses, further emphasizing the intricacies within certain solar systems.
The presence of Neptunian and terrestrial classifications among these case studies expands our comprehension of what can exist in proximity to white dwarfs. For instance, observational data points indicate a significant fraction of exoplanets residing within the habitable zone, suggesting potential environments for life. These discoveries illustrate not only the diversity of planetary formations but also the underlying dynamics that govern their evolution.
Overall, the case studies discussed here emphasize the importance of continued exploration and analysis of exoplanet discoveries which could ultimately reshape our understanding of planetary evolution in the cosmos.
The Role of Heavy Elements in Planetary Systems
Heavy elements play a crucial role in the formation and evolution of planetary systems, particularly those surrounding dead stars like white dwarfs. Observations have revealed that many giant exoplanets exhibit significant enrichment in heavy elements, possessing several tens or even hundreds of Earth masses. Such findings suggest that the process of planet formation is highly influenced by the availability of these materials.
According to core accretion models, the core mass formed before the runaway gas accretion phase is typically around 20 to 30 Earth masses. Theoretical studies indicate only a few Earth masses of heavy elements can be captured in situ during the gas accretion stage, especially within a minimum-mass solar nebula. Understanding these dynamics requires a closer look at the interaction between large exoplanets and their surrounding environments.
For example, research by Thorngren et al. (2016) highlighted a notable correlation between heavy-element mass and planet mass that merits further investigation. The enrichment model for Jupiter suggests that planetesimal accretion is essential for explaining the distribution of solids during the early solar system. Additionally, giant planets are predicted to capture tens of Earth masses of total planetesimal mass during their inward migration from a formation location a few tens of astronomical units away.
Recent observations of exoplanets, particularly those in the Panchromatic Comparative Exoplanet Treasury (PanCET) survey, provide valuable insights into these phenomena. Hot Jupiters, such as WASP-121b, demonstrate how gravitational tidal forces can significantly alter their physical shape, causing them to stretch into a football-like frustum. Temperatures in their upper atmospheres can reach approximately 4,600 degrees Fahrenheit, enabling heavy elements like magnesium and iron to escape into space, further enriching their surroundings.
Studies of these emissions enhance our understanding of how heavy elements interact within planetary systems. The metal-rich close-in Jupiters reflect a fascinating narrative of migration and formation, reinforcing the broader impact that heavy elements have on the architecture and longevity of planetary systems near dead stars.
Asteroid Dynamics in Dying Stellar Systems
The study of asteroid dynamics reveals fascinating insights into the behaviors of celestial bodies in dying stellar systems. As stars evolve and eventually transition to white dwarfs, significant gravitational interactions occur that affect the surrounding asteroid populations. Over 90% of all known exoplanets, primarily orbiting main-sequence stars, will experience this transformation as their host stars exhaust their nuclear fuel and shed mass.
During this mass loss, the dynamics of asteroids become ever more intricate. Gravitational interactions with remaining giant planets in the system can influence the migration of asteroids. These cosmic travelers may be pulled towards the central white dwarfs as they drift closer; this process opens new avenues for detecting exoplanets and debris around these stellar remnants. The implications of such migrations are crucial for understanding the survival of planetary systems post-main sequence, as highlighted in various studies on asteroid dynamics.
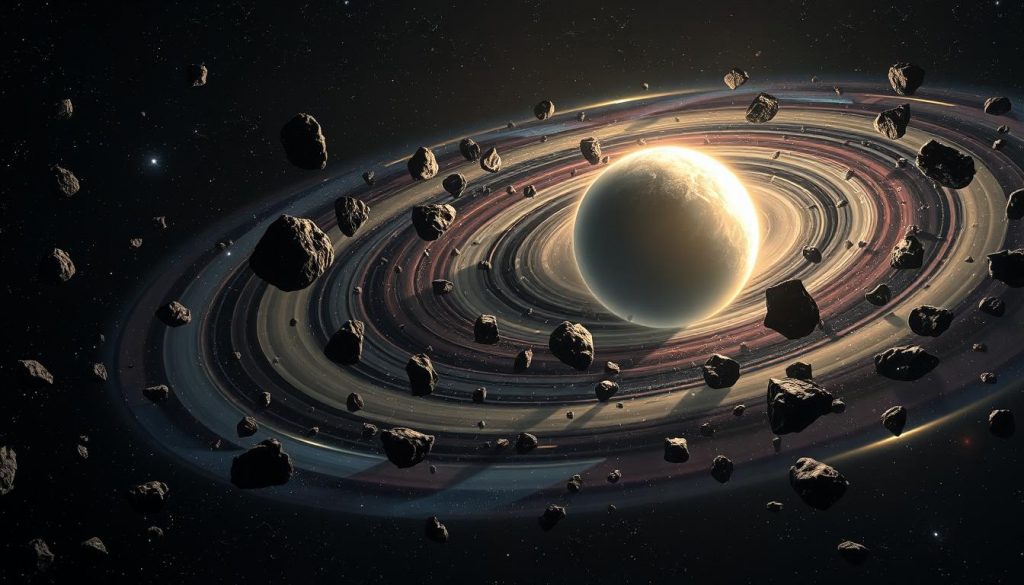
One notable aspect of this interplay is the phenomenon where the tidal radius plays a vital role in determining how long asteroids and other substellar bodies can endure before being disrupted by the white dwarf’s gravitational pull. The analysis of nearly 40 known debris discs orbiting white dwarfs enhances our grasp of these processes and offers valuable context for the broader narrative of stellar evolution.
Stellar Phase | Mass Loss Rate | Impact on Asteroid Dynamics |
---|---|---|
Main Sequence | 2.4 × 10-14 M⊙ per year | Stable orbits, minimal interaction |
Giant Phase | Increased mass loss rate | Asteroids begin to migrate |
White Dwarf | Rapid degeneration of surrounding bodies | Increased gravitational influence leads to destruction of rocky bodies |
Conducting further research in this domain not only enriches our comprehension of planetary systems but also provides crucial pathways for observing celestial phenomena. Exoplanet detection strategies can be tailored to focus on the aftermath of dying stellar systems, integrating the complexities of asteroid dynamics into modern astronomy.
For a deeper exploration of stellar evolution and its impact on habitable zones, consider reviewing this research on stellar characteristics and habitability.
Implications for the Future of Our Solar System
As we explore the fascinating dynamics of exoplanets around white dwarfs, intriguing implications arise regarding the future of our solar system. With only Earth recognized as a life-hosting planet, the prospects for planetary survival in our system warrant further examination. The evolution of our Sun holds critical importance in this narrative.
Currently, the Sun processes an astonishing 600 million tonnes of hydrogen every second. This burning of hydrogen is expected to continue for approximately 5 billion more years before transitioning into a helium-burning phase. During this transformation, the core temperature will escalate dramatically to 100 million degrees, creating extreme conditions that will profoundly affect nearby planetary bodies.
Planets like Jupiter and Saturn may face significant challenges amid the Sun’s demise. As the solar system undergoes these extensive changes, gas giants might experience shifts in orbital dynamics and environmental conditions. The future of Mars also remains a point of speculation; it was potentially habitable around 3.5 billion years ago. This history makes it intriguing as scientists consider the remnants of life that could have existed.
In a solar system rich in mysteries, the search for subsurface oceans on moons such as Europa and Enceladus fuels excitement about potential habitats. Enceladus stands out due to its active plumes, which provide insights into the moon’s subsurface without the complexities of drilling. Comparisons to worlds beyond our solar system may reveal significant patterns regarding planetary characteristics and their survival capabilities.
Key Features of Our Solar System | Future Predictions |
---|---|
Current state of the Sun | 5 billion years of hydrogen fuel remaining |
Core temperature before transformation | 15 million degrees, rising to 100 million |
Distribution of water | 99.9% exists beyond terrestrial planets |
Pivotal role of Mars | Historical habitability raises questions about past life |
Potential of Enceladus | Active sample ejection may reveal subsurface ocean chemistry |
The knowledge gained from studying exoplanets can enrich our understanding of how similar bodies might evolve within our solar system. This exploration reveals a delicate balance of planetary survival in the context of stellar evolution. As researchers examine these cosmic relationships, more insights into our solar system’s future will likely unfold.
The Search for Life Around White Dwarfs
The search for life on exoplanets orbiting white dwarfs presents a fascinating challenge for astronomers. While white dwarfs are remnants of stars that have exhausted their nuclear fuel, certain conditions may allow exoplanets in their vicinity to maintain habitable environments. Approximately 30 light-years from Earth, a mix of 74 main sequence stars, 284 red dwarf stars, and 21 white dwarfs resides. Notably, planetary systems have been identified around 60 nearby stars, yet only a few exoplanets have been confirmed around white dwarfs.
The methodologies employed in this life search primarily hinge on established detection techniques, including transit and radial velocity methods. Impressively, 98% of all exoplanets discovered utilize these two approaches. For astronomers, a mere couple of hours of observations from the James Webb Space Telescope could lead to the identification of a Jupiter-sized exoplanet in close proximity to white dwarfs. To find Earth-sized exoplanets, about 10 hours of observation is usually required among the nearest white dwarf neighbors.
Particularly intriguing is the prospect of detecting biosignatures, which may be observable around the closest six white dwarf neighbors. Each of these candidates, situated within 23 light-years, could reveal signatures of life with an estimated observing time of just 25 hours using the James Webb Space Telescope. A typical 0.6M⊙ white dwarf can harbor an Earth-like planet in its habitable zone, potentially remaining conducive to life for nearly 7 billion years.
The habitable zones of white dwarfs present a unique overlap with those of red dwarf stars such as TRAPPIST-1. This overlap creates a promising area for potential life searching as conditions may parallel those found in the early stages of Earth or other celestial bodies. In this intricate dance between stars and their planetary systems, understanding the constraints on habitability, particularly the photon fluxes needed for UV-mediated prebiotic chemistry and photosynthesis, proves crucial. Observations indicate that these requirements can be comfortably met by planets in the habitable zones around white dwarfs.
Parameter | White Dwarfs | Red Dwarf (TRAPPIST-1) |
---|---|---|
Distance from Earth | Within 30 light-years | Within 30 light-years |
Planets Discovered | Few exoplanets | Multiple exoplanets |
Habitable Zone Lifespan | Up to 7 Gyr | Recent models indicate overlap |
Observation Time for Biosignatures | Approximately 25 hours (JWST) | Significantly shorter observation time |
Photon Flux Requirement | Comfortably exceeded | Comfortably exceeded |
This extensive exploration underscores both the challenges and the fascinating possibilities presented by the ongoing life search around white dwarfs. As technology continues to advance, such inquiries into habitability may one day lead to extraordinary discoveries in our quest to understand life beyond Earth.
Future Innovations in Space Observations
As we look to the horizon, exciting advancements in space observations are on the way. With the imminent launch of the Nancy Grace Roman Space Telescope, researchers anticipate substantial leaps in our understanding of exoplanets, particularly those around white dwarfs. This innovative technology promises enhanced detection capabilities, significantly reshaping our exploration of life beyond Earth.
Current sensors such as TESS (Transiting Exoplanet Survey Satellite) access stars that are 30–100 times brighter than those previously surveyed by Kepler. TESS’s focus on small transiting planets over a planned two-year mission highlights a shift towards identifying potentially habitable worlds. On the other hand, the CHEOPS mission targets exoplanets smaller than Saturn, delivering precision measurements of radii and densities, crucial for differentiating between Earth-like planets.
The James Webb Space Telescope (JWST) stands at the forefront of telescope developments. By employing high-contrast imaging and recording spectra at infrared wavelengths, JWST will deepen our understanding of exoplanet atmospheres. Its ability to directly image young, massive exoplanets reflects a significant advancement in telescope technology innovations, paving the way for breakthroughs in planetary science.
Looking further ahead, the PLATO mission, set to launch in 2026, aims to pinpoint terrestrial planets in habitable zones around sun-like stars. This extensive exploration will include a wide sample of planetary systems, investigating their architectural nuances and evolutionary trajectories. Ariel, scheduled for launch in 2029, will conduct extensive observations of gas giants and super-Earths, building a catalogue detailing atmospheric structures across various stellar types.
With the promise of direct imaging of Earth-like exoplanets, the Habitable Worlds Observatory is projected for a 2040s launch. Integrating advanced techniques such as coronagraphy enhances our ability to research faint exoplanets, which can be up to 10 billion times fainter than their respective stars. Understanding these subtle signals requires an evolution in wavefront control technology, ensuring accurate performance during long observational sessions.
Conclusion
As we reflect on the dynamic lifecycle of stars and their remnants, the exploration of exoplanets offers profound insights into the future of Earth. With over 5,000 exoplanets discovered beyond our Solar System, we are uncovering the varied characteristics of these celestial bodies, many of which orbit white dwarfs. This phenomenon raises intriguing questions about the potential for life and habitability in fading stellar systems.
Among the discoveries, NASA’s Kepler space telescope and TESS spacecraft have each contributed significantly, identifying thousands of planets, some lurking within the habitable zone—the ideal distance where conditions may allow for liquid water to exist. Such findings encourage optimism about the possibility that life can endure, even in environments drastically different from our own.
In contemplating the unearthed data, including the detection of essential elements for life, the prospects seem promising for exoplanets as potential homes in the universe. These conclusions not only highlight our current understanding but also inspire curiosity about humanity’s own place amidst the cosmos. As we continue to explore and understand these exoplanets, we invite readers to learn more about the fascinating universe we inhabit and join the pursuit of knowledge about our stellar neighbors here.